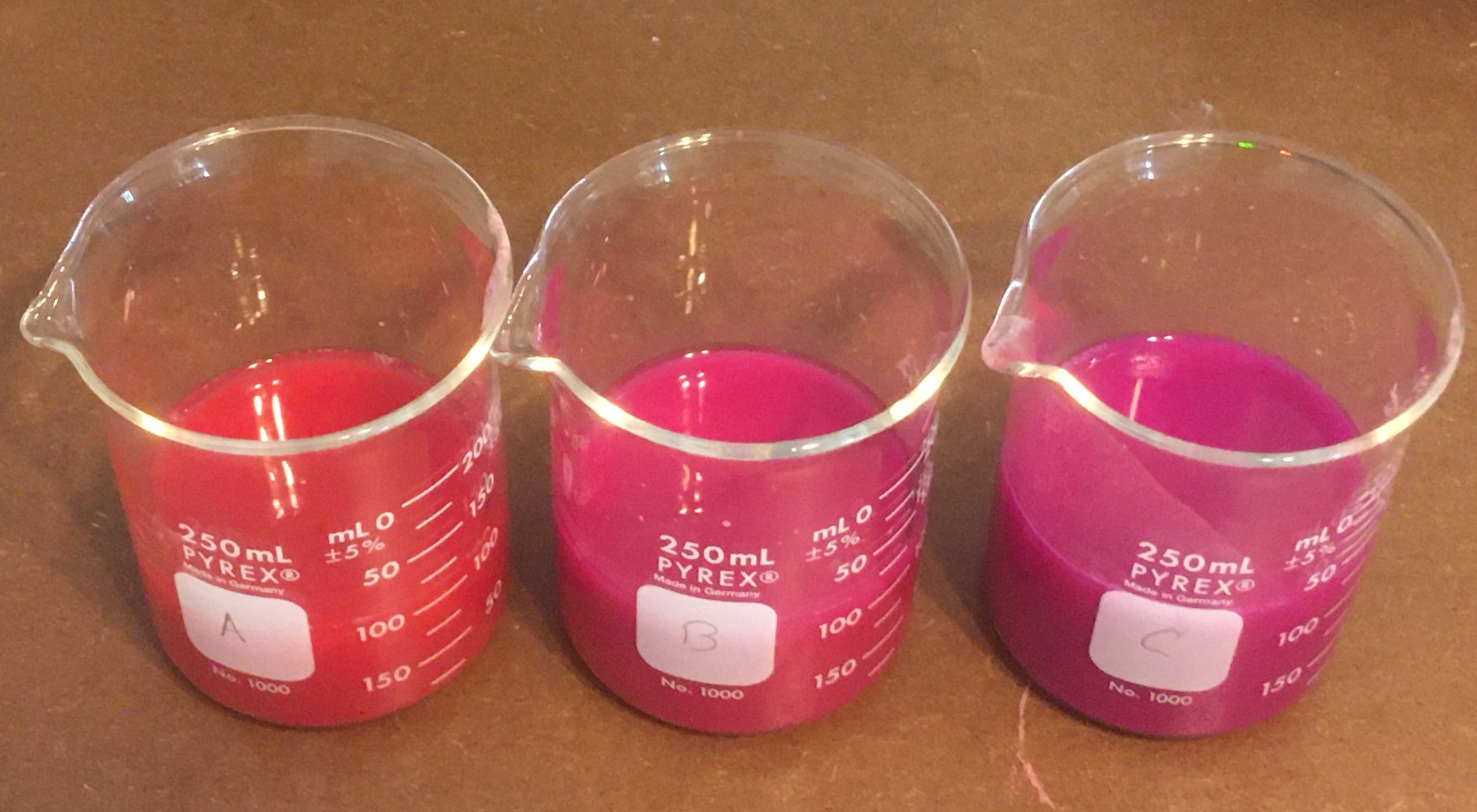
Many organic dyes, what the eighteenth-century colour-makers called “vegetable” colors, are sensitive to the alkalinity or acidity of the dyebath. I have elsewhere shared the range of bright reds and purples that can be got from brazilwood dye, by progressively adding just a few grams of mildly acidic alum; I have also repeated experiments from eighteenth-century recipe-books on the yellows of buckthorn and the purplish extract of violets. And once, while I was trying to figure out what was meant by “strong” aqua fortis, I happened upon a property of cochineal which the eighteenth-century color-makers also already knew. Instead of the bright red scarlet I was after, I got something that looked like toxic lemonade. My strong aqua fortis, in short, was much, much stronger than theirs.

For colourmakers, this property was useful for perfecting the hues of pigments. In the hands of chemist Robert Boyle, however, these secrets suggested a tool for analytical chemistry. Boyle was writing in the 1660’s, and, by his moment, certain techniques were already well known. It was well-known among alchemists that the “syrup” of violets could be transformed from red to blue by the addition of oil of vitriol, then back again, by the lees of wine. Colormakers knew that a juice extracted from turnsole—a low, shrubby annual—could be shifted using lemon juice or distilled urine; medieval illuminators depended on this trick to derive a range of colors from a single dyestuff. And he furthermore learned from the dyers that other vegetable liquors, like cornflower or privet berries, or buckthorn, cochineal, and brazilwood, could be similarly adjusted—though, in each case, each artisanal tradition depended on different additives, like alewort or fermented bran, or slaked lime or pearl ash.

Boyle’s insight was to collect all these observations in the same place—noticing that each process contained the kernel of the same sort of reaction. He moreover saw that all aqueous solutions could therefore be described in terms of their effects on these organic substances, that the effect, as it were, sorted reagents into categories. He had taken a few trade secrets and alchemical experiments, and used them to make broad observations about the invisible qualities of solutions. Boyle doesn’t seem to have been the first person to have made this leap. But his insight was nevertheless an important waypoint in the history of what would become litmus paper, that rough-and-ready tool still among the most important utensils in the chemist’s laboratory.

But what causes the color-shifting trick: that remained a mystery. Boyle knew that it only works on organic dyestuffs; it has no effect on mineral pigments, like bice or the mercuric sulfide called “vermillion.” What is more, this process is different than what happens when vegetable dyes are mordanted, as when we add alum to brazilwood in the first step of the modern laking process. While different mordants produce different effects (i.e. the difference between Dutch and Venetian Scarlet) adding a mordant triggers a transformation that happens once, and is mostly irreversible. But adjusting the pH level of a dyebath can shift the color in ways that can be easily reversed: a few milliliters of muriatic acid, for instance, nudges a brazilwood dye towards blue, but a gram or two of alkaline pearl-ash prods it back toward the red.
Here’s where this post gets “wonky,” as the economists say, as in, “preoccupied with arcane details or procedures in a specialized field.” Skip the next four paragraphs if you’d like to get to the punchline.

Boyle speculated that acids and bases “insinuated themselves into the pores” of the “tingeing particles,” thus changing the “asperity” of their surface (and their reflectance properties). That’s a good start. But with the advantage of insights made possible by Boyle’s analytical tool, we can now sharpen up our sense of what’s going on, in a molecular sense. An acid is a liquid which, slightly counterintuitively, is prone to donate protons; although acids burn, and can sort of melt things like metals away in to liquid, they are actually, at a molecular level, just very, very aggressive donors. An acid has an excess of protons, and when another substance is dissolved in it, it is especially active in trying to deliver its extra positive charge. An alkali is the opposite; it is a liquid which wants to pry protons away from anything dissolved in it. It is for this reason that acids and alkalis neutralize each other: acids donate protons which alkalis gladly accept.
Organic dyes are large chains of carbon rings, studded with extra protons in the form of hydrogen (H) or hydroxyl groups (-OH); they are, therefore, particularly apt to be modified by acids and alkalis, since those rings can accept more protons, or the ones it already has, can be cracked off. Put an organic molecule in an alkaline dyebath, and it cracks some of those limbs off. Put in an acidic one, and it adds a few extra. In either case, the total structure of the whole molecular compound is subtly changed.
Now: when a photon, the smallest quantum of light, strikes a dye molecule, it is absorbed by an electron; that extra energy shifts it into a higher orbit. But the electron can only stay in that higher orbit briefly—and when it finally collapses back into its lower, more comfortable state, it sheds a photon in compensation, which is of a wavelength exactly equal to the energy it loses. In the case of single atoms, these wavelengths can be calculated based on the structure of the nucleus and its electrical charge. But in the case of organic compounds, the effects get complex. Because carbon is such a good conductor, some of the electrons of an organic compound are shared across the total molecular structure. When a photon strikes that molecule, therefore, it excites an electron which is part of that whole cloud-like field; and when that cloud sheds that energy, it is one electron somewhere in the whole cloud which emits that photon.
Changing an organic compound, therefore, by adding or stripping away a proton, effects subtle changes in the whole molecule’s total reactivity. Stripping away a proton means that the whole compound has more of those relatively free-floating electrons; the molecule becomes more reactive, and therefore liable to release higher-energy, shorter wavelength photons. Shorter wavelengths shift towards blue. Conversely, adding a proton—by putting it in an acidic environment, for instance—renders the whole compound relatively electron-poor, in the sense that there are fewer electrons that aren’t tightly bound to protons. This produces a less-reactive system, which will emit lower-energy photons. Lower energy means longer, slower wavelengths: more red.
This, then, is the punchline, the explanation of that color-shifting effect. An acidic solution makes the dye molecule less reactive. The photons it emits are less energetic: more red. The alkaline one makes it more reactive. The photons it emits are more energetic: more blue. Now, it isn’t quite as simple as this; the colors we see don’t simply slide along a spectrum, for reasons that partly have to do with how the eye perceives colors. But the direction of the shift with organic dyes is mostly consistent: acidic is towards red, and alkaline, towards blue.

I mention this all at some length—the color shifting trick, and its relationship to the pH scale—in part because I’ve done this research, and written up these findings, under funding by the Beckman Center for the History of Chemistry, which is (in effect) pH money. Arnold Beckman made his first fortune developing the portable analytical tool which superseded Boyle’s invention—and it is largely from that device, and the company Beckman founded to build analytical instruments, that the Beckman Center received its major endowment. Exactly two floors beneath my carrel is the public wing of the same institution, the Science History Museum, where hangs the so-called “Shannon” portrait of Robert Boyle. Here, a certain historical circuit is completed– or, I mean, *almost* completed; Boyle, flanked by his major publications, gazes serenely down on exhibits which include the history of modern dyestuffs, and on the early history of chemical instruments. It is one of the great disappointments to anyone interested in the history of pH instruments that Boyle’s effigy cannot gaze upon Beckman’s instrument; his eyeline is blocked by a concrete pillar, which no amount of alchemical tricks have yet succeeded in making transparent.